Fluorescence Lifetime Measurements using Entangled-Photon Coincidences
Authors:
Audrey Eshun* ([email protected]), Xiyu Yi, Ashleigh Wilson, Sam Jeppson, Shervin Kiannejad, Mike Rushford, Tiziana Bond, and Ted Laurence
Institutions:
Lawrence Livermore National Laboratory
Abstract
Researchers have utilized entangled photon pair coincidences to measure the fluorescence lifetime of the organic dye Rhodamine 6G, allowing lifetime measurements to be conducted at low intensities with a continuous wave (CW) laser and without ultrafast pulsed lasers.
Quantum entangled photons have been studied over the years for their strong correlations and potential for novel applications in imaging, communication, and computing. In the realm of imaging and spectroscopy, these correlations allow measurements to be made that may offer increased information, as well as to be conducted with much lower light intensities, which is advantageous in the imaging and spectroscopy of sensitive materials. With these goals in mind, researchers have generated a high flux of entangled photon pairs using spontaneous parametric downconversion (SPDC) and with this, have measured the fluorescence lifetime of the organic dye Rhodamine 6G, utilizing entangled photon pair coincidences, conducting these measurements at low intensities, with a CW laser and without the use of ultrafast pulsed lasers. This differs from alternative methods of obtaining fluorescence lifetimes without pulsed or modulated lasers, such as the use of antibunching, which requires significantly longer integration times.
Ghost imaging, which involves using one photon to probe an object in front of a bucket detector with the other photon serving as a reference to reconstruct the image has been widely demonstrated and proven to work (Pittman et al. 1996; Padgett and Boyd 2017). Many experiments utilizing the concept of ghost imaging have been theorized, such as a method hypothesized by Scarcelli and Yun (2008) for using fluorescence excited by entangled photons for imaging with coincidence counts; one photon is absorbed by the sample, producing fluorescence, and the other holds information on where that absorption took place. The ability to successfully measure the fluorescence induced by low intensity excitation is critical towards achieving such fluorescence imaging and can be further exploited by using the time correlations of the photon pair to measure the fluorescence lifetime of the substance being studied. The signal photon acts as a probe, excites the sample, and causes emission of a fluorescence photon, which is measured by a single photon detector. A delayed time correlation exists between the idler photon at a reference detector and the fluorescence photon; thus, coincidence counts can be measured between them when fluorescence occurs and a plot of coincidences against time delay will reveal a correlation peak whose decay time is the fluorescence lifetime of the sample. Lifetime measurements are usually carried out with the time correlated single photon counting method, using pulsed lasers or alternatively a form of modulated excitation. The entangled photon method achieves these measurements with a continuous wave laser and runs with 1 minute integration times.
Entangled photons centered around 532nm were generated using Type-I spontaneous parametric down conversion (SPDC). A 266nm continuous wave laser (Topwave 266-300, Toptica) was used to pump a 1mm thick BBO crystal cut for degenerate Type-I SPDC [NCBBO5100-266(1)-A, Newlight Photonics]. Harmonic beam splitters (Y4-2037-45, CVI Laser Optics) filter out any residual 532nm from the third harmonic generation of the 266nm pump. The pump power can be attenuated by rotating a half wave plate (HWP [WPH10M-266, Thorlabs]) placed in front of a Glan prism polarizer (GLB10-UV, Thorlabs) before being passed through a narrow bandpass filter (ET270/15BP, Chroma) to ensure a clean 266nm centered pump. The pump passes unfocused through the BBO crystal and then through a 35mm focal length collimating lens (LA4052-UV, Thorlabs). After the crystal and collimating lens, a dichroic mirror (355nm reflection cutoff; Di01-R355-25×36, Semrock) separates the remaining 266nm pump from the generated SPDC light, reflecting the pump into a beam dump. A wide bandpass interference filter (545QM75, Omega Optical) further cleans the SPDC emission from any spurious pump light. Photons of an entangled photon pair have corresponding energies that add up to that of the pump. Therefore, the signal and idler photons can be separated via wavelength, as each photon on one side of the SPDC center wavelength has a corresponding photon on the opposite side. A dichroic mirror cut near 532nm (FF538-FDi02-t3-25×36, Semrock) transmits all light 532nm and above to create the idler path and reflects all light 532nm and below to create the signal path.
The idler path acts as a reference while the signal path is the excitation arm. An optical relay is constructed in the signal arm with two 250mm lenses (AC254-250-A-ML, Thorlabs) to ensure the beam profile of the SPDC ring is clearly conjugated onto the back aperture of the excitation objective. A dichroic mirror (Di02-R532-25×36, Semrock) reflects the signal beam into an objective lens, and the sample is held above the objective in a glass bottom dish. Two objective lenses, a 20x 0.42 Numerical Aperture objective (M Plan Apo 20x 378-804-3, Mitutoyo), and a 25x 1.1 Numerical Aperture water immersion lens (N25X-APO-MP 25x, Nikon) were used interchangeably in this study. The resulting emission is collected via the same objective and transmitted through the dichroic mirror and emission filter and focused on the signal detector. The two photon counting detectors (PMA Hybrid 40 Photomultiplier Detector, Picoquant) are connected to a time controlling device (ID900, IDquantique) to measure coincidence counts.
The measurement in Fig. 1 shows the fluorescence lifetime decay of a 100mM solution of Rhodamine 6G. These measurements were conducted with 3 different concentrations (100, 10 and 1 𝜇𝑀; all graphs not shown) of the dye and with two objectives with varying numerical apertures (NA). As expected, the measured coincidence counts increase with objective NA and with dye concentration, confirming the fluorescence origin of the measured signal. Ethanol is used as a control and shows background level coincidence counts and no correlations (Fig 1). Fitting the decay of the measured curves provides the fluorescence lifetime and researchers obtained lifetimes of 3.7ns, which agrees with previously measured fluorescence lifetime values for this dye (Beaumont, Johnson, and Parsons 1993; Magde, Wong, and Seybold 2002). With this study, researchers have successfully shown that fluorescence lifetime can be measured at low intensities with entangled photons, without pulses using a CW laser.
Image
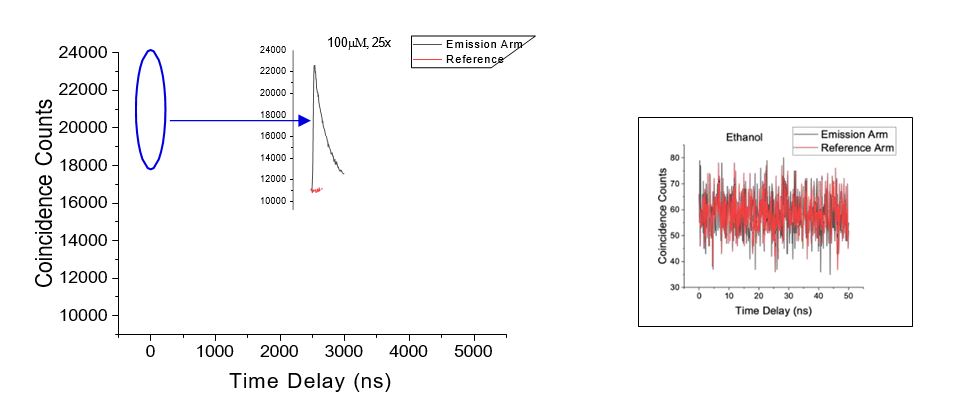
Fig 1. Correlation graphs showing coincidence counts as a function of time delay for (left) Rhodamine 6G at 100M (inset shows zoomed in spike at shorter timescales) and for (right) ethanol showing no coincidences.
References
Pittman, T. B., et al. 1996. “Two-Photon Geometric Optics.” Physical Review A 53(4), 2804–15.
Padgett, M. J., and R. W. Boyd. 2017. “An Introduction to Ghost Imaging: Quantum and Classical.” Philosophical Transactions of the Royal Society A: Mathematical, Physical and Engineering Sciences 375(2099).
Scarcelli, G., and S.H. Yun 2008. “Entangled-Photon Coincidence Fluorescence Imaging.” Optics Express 16(20), 16189–94.
Beaumont, P. C., D. G. Johnson, and B. J. Parsons. 1993. “Photophysical Properties of Laser Dyes: Picosecond Laser Flash Photolysis Studies of Rhodamine 6G, Rhodamine B and Rhodamine ” Journal of the Chemical Society, Faraday Transactions 89(23), 4185–91.
Magde, D., R. Wong, and P. G. Seybold. 2002. “Fluorescence Quantum Yields and Their Relation to Lifetimes of Rhodamine 6G and Fluorescein in Nine Solvents: Improved Absolute Standards for Quantum Yields.” Photochemistry and Photobiology 75(4), 327–34.
Funding Information
This work was performed under the auspices of the U.S. Department of Energy by Lawrence Livermore National Laboratory under Contract DE-AC52-07NA27344.